We selected two reactions to assess the ability of the CSTR cascade to handle the formation of solids: (i) reaction of glyoxal with cyclohexylamine to form N,N′-dicyclohexylethylenediimine that is insoluble in the reaction solvent, ethanol (Scheme 2),19 and (ii) sulfonylation of 2-octanol (Scheme 3),43 for which the side product, triethylamine hydrochloride, has limited solubility in the solvent, dichloromethane (DCM).43

Scheme 2 Imine formation reaction of glyoxal and cyclohexylamine.
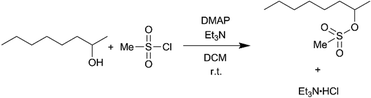
Scheme 3 Sulfonylation of 2-octanol with methanesulfonyl chloride.
The first reaction (Scheme 2) tested the ability of the CSTR cascade to handle the formation of a solid product at relatively high solid loadings, 4.4% (wt), corresponding to stoichiometric amounts of the reagents and a glyoxal concentration of 0.4 M. The reaction reached nearly 100% conversion in 15 min with a 6-unit CSTR cascade. Adding more CSTRs after the 6-unit cascade would not lead to the growth of particles since the reaction was complete and, instead, help rebalance the particle size distribution. A total flow rate of 1 ml min−1, rapid stirring (∼600 RPMs), and short connections between adjacent chambers allowed transport of solid particles inside the CSTR cascade without clogging (Fig. 5). However, gravity caused particles to accumulate in the outlet tube. To avoid this problem and enable facile flow of particles through the entire system, the CSTR cascade was positioned vertically to align the gravity force and the outlet flow direction. As the reaction proceeded, the particle concentration in each chamber increased along the flow direction (Fig. 5).
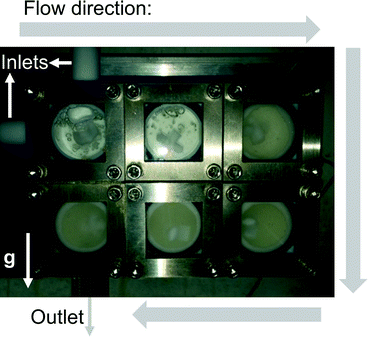
Fig. 5 Photograph of the CSTR cascade during the operation showing the solid fraction increasing along the flow direction (the white arrow marked g shows the direction of gravity).
Pressure measurement at the reactor inlet was a sensitive indicator of potential clogging problems. If particles built up in the reactor and clogged the flow path, the relative pressure would rise dramatically without going back. The system automatically would stop the pump to avoid damage to the pump or reactor when the pressure exceeded 10 bar. The pressure profile (Fig. 6a) demonstrates that the system could run continuously for 24 h without significant signs of clogging. Besides small pressure fluctuations in the reactor, a pressure spike occurred around 1000 min reflecting minor particle accumulation at the outlet and the solid clusters being pushed through by the elevated pressure. Once the particle clusters had left the system, the pressure returned to the base line. The solid product was an organic crystal, which had a slice-shaped morphology (Fig. 6b) and an average size of 226.7 μm with a standard deviation of 108.7 μm (Fig. 6c). Even though particles with sizes over 500 μm existed in the outlet stream, the larger interconnections (3.2 mm) and the limited amount of very large particles meant that they could flow through the interconnections without problems.
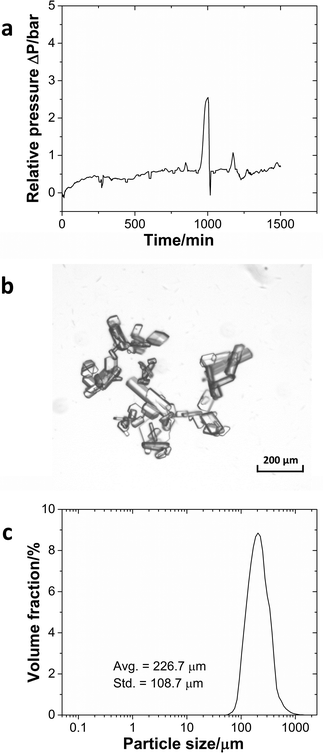
Fig. 6 (a) Pressure profile during the continuous operation of the imine formation reaction. (b) Microscopy image of the imine crystal. (c) The particle size distribution of the product.
In the previous case, the solid particles were the main product of the reaction. In the next example, sulfonylation of 2-octanol (Scheme 3), the side product, triethylamine hydrochloride, has limited solubility in the solvent, dichloromethane (DCM).43Moreover, the crystals are needle-shaped (Fig. 7a) and thus agglomerate easily. When feasible, needle-shaped crystals are avoided in the pharmaceutical industry due to their poor flow properties. A 3-unit CSTR cascade sufficed to obtain full conversion at a flow rate of 1.00 ml min−1 of 0.6 M 2-octanol and 0.72 M methanesulfonyl chloride. Even with a solid loading of 4.1% (wt), the CSTR cascade ran continuously for 8 h without clogging, as reflected by the pressure profile (Fig. 7b).
Fig. 7 (a) The microscopy image of the triethylamine hydrochloride salt. (b) Relative pressure profile during the continuous operation of the sulfonylation reaction.
Conclusion and perspectives
The case studies demonstrate the ability of the CSTR cascade to process reactions containing solids continuously. The reactor assembly, consisting of PTFE reactor chambers, glass and stainless steel covers, has excellent chemical resistance for most chemical reactions and is easy to clean after usage. The chambers’ mount makes it easy to vary the number of units in the CSTR cascade. The homogeneous concentration and temperature profiles realized by strong agitation in each chamber result in nearly ideal CSTRs in series RTD profiles and accurate predictability of reaction conversions. Moreover, the high rate of stirring keeps the particles suspended in each reactor chamber, preventing them from sticking to the reactor wall or agglomerating. The short distance between adjacent reactor chambers minimizes the possibility of clogging at connections. Running the CSTR cascade in a vertical mode so that gravity aids particle transport out of the reactor eliminates clogging of the outlet tube. These rational design aspects contribute to the capability of long-time continuous handling of solids in the reactor. For different types of solid-forming reactions, such as rapid solid formation with fast growth kinetics, a careful investigation of suitable reaction conditions (e.g. reagent concentrations, reaction temperature, flow rates, etc.) needs to be performed in order for these reactions to proceed in the CSTR cascade.
The capability to handle solids also enables its application to continuous crystallization, where tuning different operational parameters (e.g. rotation speed of stir bar and flow rates) can control the morphology of crystals in the CSTR cascade. The scope of the miniature CSTR cascade could be expanded by giving each unit a set of functions, such as independent temperature control and multi-injection points. Such modular units would enable telescoped multistep reactions in a single miniature CSTR cascade. Furthermore, the strong agitation in each chamber would also be beneficial for creating large contact areas and the resulting mass transfer for liquid–liquid reactions.